Cranium Conundrum Creek
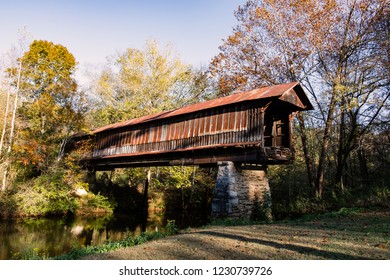
Make it happen.
The integration of fossils, phylogeny, and geochronology has resulted in an increasingly well-resolved timetable of evolution. Life appears to have taken root before the earliest known minimally metamorphosed sedimentary rocks were deposited, but for a billion years or more, evolution played out beneath an essentially anoxic atmosphere. Oxygen concentrations in the atmosphere and surface oceans first rose in the Great Oxygenation Event (GOE) 2.4 billion years ago, and a second increase beginning in the later Neoproterozoic Era Neoproterozoic Oxygenation Event (NOE) established the redox profile of modern oceans. The GOE facilitated the emergence of eukaryotes, whereas the NOE is associated with large and complex multicellular organisms. Thus, the GOE and NOE are fundamental pacemakers for evolution. On the time scale of Earth’s entire 4 billion–year history, the evolutionary dynamics of the planet’s biosphere appears to be fast, and the pace of evolution is largely determined by physical changes of the planet. However, in Phanerozoic ecosystems, interactions between new functions enabled by the accumulation of characters in a complex regulatory environment and changing biological components of effective environments appear to have an important influence on the timing of evolutionary innovations.
On the much shorter time scale of transient environmental perturbations, such as those associated with mass extinctions, rates of genetic accommodation may have been limiting for life. INTRODUCTIONDeep time and its codification in the geologic time scale stand as the intellectual triumph of 19th century geology. Initially, time was marked by the comings and goings of fossils, a relative time scale recognized, after Darwin, as the historical record of evolution. However, with the discovery of radioactivity, the prospect of calibrating geologic time in years arose. In 1907, Arthur Holmes used Bertram Boltwood’s research on the radioactive decay of uranium to date ancient terrains in Sri Lanka at 1640 million years, and soon thereafter, Joly and Rutherford argued from pleochroic halos in granite that Devonian rocks are at least 400 million years old (Ma). Despite this, routine application of radiometric dating to Earth history accelerated only half a century later, in conjunction with better instruments and careful mapping of Earth’s oldest rocks.
The calibration of evolutionary history requires that paleobiological and biogeochemical evidence be integrated with accurate and precise geochronology within a spatial framework provided by careful mapping and measured stratigraphic sections. The result is not only a timetable of evolution but also an improved sense of evolutionary rates as they have varied through time. Here, we review applications of increasingly sophisticated geochronological methods that have altered our sense of evolutionary pattern, including both radiations and extinctions. We can identify evolutionary events as fast or slow, but relative to what? Can evolutionary theory provide predictions that can be tested against the empirical record? Is the pace of evolution inferred from geologic history governed largely by genetics, or does environmental change beat the evolutionary tattoo?
With these questions in mind, we compare theoretical approaches to evolutionary time scale with the record reconstructed from sedimentary rocks. Radiations through Earth historyTo begin at the beginning, we can ask when life first gained a persistent toehold on Earth. Following the discovery of microbial fossils much older than the oldest known animals , paleontological and biogeochemical attention turned quickly to Earth’s oldest little metamorphosed sedimentary rocks. Radiometric dates soon established that the thick sedimentary-volcanic succession of the Swaziland Supergroup, South Africa, was older than 3 billion years , but geologic relationships between dated granites and biologically informative sedimentary rocks remained uncertain and analytical uncertainties were large. The evolutionary timetable, showing the course of evolution as inferred from fossils, environmental proxies, and high-resolution geochronology.Phanero, Phanerozoic; Prot, Proterozoic; Ceno, Cenozoic; E, Ediacaran; Cam, Cambrian; O, Ordovician; S, Silurian; D, Devonian; Car, Carboniferous; Per, Permian; Tr, Triassic; J, Jurassic; K, Cretaceous; Pal, Paleogene; Neo, Neogene. Crosses indicate times of major mass extinctions.A second milestone in the history of life was the initial rise of oxygen in the atmosphere and surface oceans, an event known as the Great Oxygenation Event (GOE) ( and ). An environmental transition of key biological importance, the GOE is recorded geologically and geochemically, most notably (and quantitatively) by the end of large mass-independent sulfur isotope fractionation in sedimentary sulfides and by the last appearances of redox-sensitive minerals as detrital grains in sedimentary rocks.
South African rocks that record the S-isotopic shift lie above volcanic beds dated by SHRIMP U-Pb zircon geochronology at 2480 ± 6 Ma and below pyritiferous shales dated by Re-Os methods at 2316 ± 7 Ma. The last appearance of redox-sensitive detrital minerals is constrained to be younger than 2415 ± 6 Ma by a SHRIMP U-Pb date for zircons in an underlying ash bed , whereas recent analysis of an overlying volcanic unit yields a date of 2426 ± 1 Ma. Thus, geochronology and geochemistry together place sharp constraints on the timing of the GOE and, therefore, the minimum age of oxygenic photosynthesis and the diversification of oxygen-requiring metabolic and biosynthetic pathways. Photo credits: All photos by Andrew H.
Knoll.Moving forward in time, the record grows richer, calibrated by high-resolution U-Pb analyses of zircons from ash beds interbedded with biologically informative rocks and, increasingly, Re-Os analyses of carbonaceous shales that actually contain key paleobiological records. Eukaryotic cells evolved phagocytosis, increasing the role of predation in microbial ecosystems; protists also established a genetic and cell biological foundation for the later evolution of complex multicellularity. The oldest microfossils of probable eukaryotic affinities are large (100 μm), faintly ornamented vesicles from the Changzhougou Formation, China. Conventionally described as 1800 to 1600 Ma, Changzhougou fossils are now constrained to be younger than the 1673 ± 10–Ma U-Pb age of zircons in a granite-porphyry dike that cuts underlying rocks and older than the 1625.3 ± 6.2–Ma ash bed in overlying beds. Shales (1600 to 1400 Ma) distributed globally contain similar assemblages of moderately diverse microbial eukaryotes reviewed by Javaux and Knoll as well as simple multicellular forms (, ), but the oldest fossil population reliably assigned to a crown group eukaryotic taxon comprises simple multicellular red algae preserved in silicified carbonates from Arctic Canada only loosely constrained to be ca.
1200 to 1100 Ma.The eukaryotic fossil record expands to include vase-shaped protistan tests , mineralized scales , and an increasing number of ornamented cell walls and coenocytic taxa (, ) in rocks deposited during or after the Bitter Spring carbon-isotope anomaly, constrained by an 811.51 ± 0.25–Ma ash bed just below its onset. Increasing time resolution in Neoproterozoic paleontology is exemplified by taxonomically similar multispecies protist assemblages formed 4000 km apart but of indistinguishable age, within the resolution of radiometric dating.
Molecular clocks suggest that animals were part of the mid-Neoproterozoic diversification of eukaryotes , although animal body fossils occur only in younger rocks. In addition, later Neoproterozoic rocks record renewed redox transition, sometimes christened the Neoproterozoic Oxygenation Event (NOE) ( and ). Some evidence, for example, from selenium isotopes , suggests that the NOE began as early as 750 Ma, whereas diverse geochemical data indicate Ediacaran acceleration and still other proxies (Fe speciation and Mo isotopes) suggest that the permanent oxygenation of deep oceans occurred only within the Paleozoic Era (, ). The precise nature and timing of this transition remains a subject of research, reflecting incomplete and sometimes contradictory geochemical results, limited radiometric calibration, insufficiently appreciated diagenetic influences, and, very possibly, a complex temporal pattern of oxygen fluctuation before a new steady state was achieved. What is clear is that the NOE encompasses the initial radiation of large, complex animals. The diversification of animals occurred within the context of the NOE, likely reflecting both new physiological opportunities attendant on increasing PO 2 (partial pressure of oxygen gas) and, possibly, feedbacks from emerging animals onto marine redox profiles.From our current perspective, it might seem surprising that 25 years ago, there was no consensus on the age of the Proterozoic-Cambrian boundary or of rocks on either side of the boundary that preserve fossils of early animals. The fact that we have an increasingly refined temporal framework for early animal diversification today owes much to the work of Samuel Bowring.
Isotope dilution thermal ionization mass spectroscopy (ID-TIMS) provides U-Pb ages of unprecedented accuracy and precision and is especially useful when zircons have experienced a complex geological history. Modern attempts to construct a timetable of early animal evolution began with ID-TIMS dating of zircons from a volcanic breccia intercalated among Siberian siltstones that contain basal Cambrian skeletal fossils. The published date of 543.9 ± 0.25 Ma has since been complemented and refined by U-Pb dates from ash beds in Namibia, Oman, and China, leading to a consensus age of 541 ± 1 Ma for the Proterozoic-Cambrian boundary (–).
Fossils record the sequential evolution of bilaterian body plans and diversity during the first half of the Cambrian Period, with much of the action taking place after 525 Ma (, ); however, the soft-bodied fossils that illuminate Cambrian evolution with exceptional brightness are only moderately well placed in time. Because none of these are directly constrained by radiometric dates, their ages are estimated on the basis of biostratigraphic correlation with successions that contain dated volcanic rocks. On this basis, the Sirius Passet fauna in Greenland is considered to be about 520 Ma; the remarkably diverse Chengjiang fauna in China is perhaps a few million years younger, and the Emu Bay fauna in Australia is a bit younger than that. All are older than 514.45 ± 0.36 Ma. The Burgess Shale, most famous of all exceptionally preserved Cambrian faunas, is constrained to be younger than 510 ± 1 Ma and older than 503.14 ± 0.13 Ma.
Collectively, then, fossils tell us that diverse animal body plans emerged during the first 30 million years of the Cambrian Period, with stem group members of extant phyla and classes dominating marine faunas.Looking backward from the Proterozoic-Cambrian boundary, the oldest known animal body fossils, osmotrophic metazoans, ascribed to early branching, occur in rocks constrained from below by a 570.94 ± 0.38–Ma ash bed and from above by another ash, dated at 566 ± 0.35 Ma , with greater body plan diversity emerging only later. Trace fossils interpreted in terms of bilaterian locomotion also occur in ca. The timing and rapidity of mass extinctionsThe fact that diversity declined and faunas changed markedly at discrete times in Earth history was well known to 19th century scientists ; the most prominent of these changes—now understood to reflect mass extinctions—dictated the subdivision of Phanerozoic time into the Paleozoic, Mesozoic, and Cenozoic eras. Despite this, mass extinction figured only peripherally in discussions of evolutionary history until 1980, when Alvarez et al. hypothesized that bolide impact at the end of the Cretaceous Period devastated the planet, eliminating nonavian dinosaurs and many other taxa.
The Alvarez paper was a hypothesis about mechanism but also about timing and rate. Chemical, mineralogical, and sedimentological features attributable to impact were identified in extinction-level strata, and subsequently, a massive crater was recognized beneath the current surface of the Yucatan Peninsula. Critically, 40Ar/ 39Ar dates on Haitian tektites generated by the Chicxulub impact are essentially indistinguishable from those on a bentonite 80 cm above the mass extinction horizon in Montana: 66.038 ± 0.25 Ma versus 0.66.019 ± 0.021 Ma, respectively. Thus, geochronology provides key support for the Alvarez hypothesis, and conversely, it is accurate and precise geochronology that keeps Deccan Trap volcanism in the discussion of biologically important geophysical changes near the Cretaceous-Paleogene boundary (, ).Phanerozoic rocks record five episodes of major diversity decline, along with a dozen or more smaller extinction episodes.
Early optimism that impact might provide a general mechanism for mass extinction faded; however, to date, only end-Cretaceous extinction is securely tied to bolide impact. In contrast, massive volcanism has emerged as the leading candidate for at least two major extinctions. Notably, Siberian Trap volcanism is widely accepted as the trigger for the largest known extinction event, at the end of the Permian Period. U-Pb zircon dates for ash beds in China that bracket the extinction constrain its timing to between 251.941 ± 0.037 Ma and 251.880 ± 0.031 Ma , indistinguishable from a 251.901 ± 0.061–Ma U-Pb date for the major phase of Siberian Trap eruption. Thus, geochronology establishes the synchroneity of volcanism and extinction, but it also does more, emphasizing the rapidity of the extinction and the events that surrounded it. In principle, massive volcanism could drive extinction via volatile release, leading to global warming, ocean acidification, and oxygen depletion in subsurface ocean waters. However, for these mechanisms to work, the time scale of emission must be fast (, ).
The Siberian traps pass this test, thereby providing both a trigger and physiological kill mechanisms for end-Permian extinction.Increasing evidence now links end-Triassic mass extinction with massive volcanism as well , and at least some minor extinctions during the Mesozoic Era also coincide with massive volcanism. An unanswered question relevant to our environmental future is why some massive volcanic events caused mass extinction, whereas others did not.
In any event, the conclusion that CO 2 is currently increasing at rates not seen during the preceding 66 million years should give us pause. A THEORETICAL FRAMEWORK FOR THE EVOLUTIONARY TIMETABLEEvolutionary theory can make statements about the time scales associated with evolutionary dynamics. The results that are best understood concern the rates at which individual mutations occur and reach fixation or become extinct. For example, we can estimate the time scale on which populations of viruses, bacteria, or cancer cells accumulate point mutations that confer resistance to treatment (, ), or we can estimate the times until microbial populations discover adaptations based on individual point mutations or other small genetic alterations (–).
But what can we say about the longer evolutionary time scales that are relevant for the events reviewed here? How long would it take for a planet of bacteria to discover the tools required for oxygenic photosynthesis or a world of prokaryotes to construct eukaryotes by endosymbiosis?Today, an estimated 10 30 bacteria populate Earth. Most of these cells live in the ocean, where they divide, on average, every 1 to 10 days. If these estimates hold for the past, the billion years recorded by Archean sedimentary rocks should have experienced about 10 41 cell divisions. On early Earth, population sizes and reproductive rates might well have been different, but even if modern estimates are off by several orders of magnitude, the number of Archean cell divisions must still have been impressive. What can evolution discover with this reproductive potential, and how long would such a process take?Progress toward answering such a question depends on our understanding of the mechanisms by which evolution discovers new functions.
Evolutionary innovations lead to qualitatively new phenotypic traits. They can be caused, for example, by new genes, new combinations of genetic material, new regulatory circuits, and new metabolic networks (–). In the world of complex organisms, evolutionary innovation could be the emergence of a new organ or a new tissue or the expression of a gene in a novel cell type. Here, we adopt the perspective that the fundamental basis of every evolutionary innovation is a modified genetic sequence.
Even phenotypic plasticity is ultimately encoded genetically. In the following, we mostly consider evolutionary innovation in the world of prokaryotes.Evolution is a search process. The search occurs in the high dimensional space of genetic sequences.
The steps in this space include point mutations, insertions, deletions, and genetic rearrangements. Evolutionary innovation can include fusion or amplification of genes, of parts of genes, bringing together distinct genes in a single cell and bringing the expression of a gene under the control of new promoter. Imagine that a new function, not currently present in a bacterial population, requires a new gene of length L. The average bacterial gene is L = 1000 nucleotides long, so one possibility to imagine the search for new function is to consider a bacterial population exploring the hypercube of sequence space containing L dimensions and 4 L sequences.
Attainment of a new function will depend on the structure of the existing protein encoded by the gene. Because there is redundancy on the level of the genetic sequence, any particular protein structure can be encoded by many different genetic sequences. Therefore, the searching population would not be required to find a unique sequence. In principle, the space of 4 L sequences will contain many solutions, and these solutions might be widely distributed in sequence space. Nevertheless, one can show that the search for solutions of L = 1000 can quickly exhaust the evolutionary potential of an entire planet unless the solutions are so abundant that even random sequences might contain them with high probability.
The straightforward evolutionary search leads to a strongly dichotomous result: (i) The solution is hyper-abundant so that random sequences can solve the task or (ii) the solution will almost certainly not be found by an entire planet of bacteria searching for a billion years (or more).How then does evolution discover new functions whose target sequences are not hyper-abundant? One mechanism that can solve the problem is the “regeneration process,” in which the genetic machinery of the population regenerates starting sequences by means of gene recombination and/or duplication that are a certain number of steps, say k, away from a target sequence. Those steps can include point mutations, insertions, deletions, and genomic rearrangements. It could be that some steps need to occur in a particular order, whereas others can occur in any order.
Therefore, the regeneration process can include epistatic interactions between mutations and potentiating mutations. We envisage the regeneration process as a sequence of steps that are not individually favored by natural selection. Only the final product is favored by natural selection. If individual steps are already favored, then the evolutionary dynamics would describe the stepwise improvement of an existing function rather than the discovery of a new function.
The incremental adaptation would certainly occur quickly on a geological time scale. The regeneration process.Gene duplication ( A) or recombination ( B) generates a starting condition for the search process, at rate w. ( C) From the starting condition, we require k mutational steps, each at rate u, to reach the target sequence, which encodes a new function.
At each step, there is the possibility to receive inactivating mutations, at rate v, which destroy the search. The frequency of the wild type is denoted by x 0. The frequencies of the intermediate steps in the search process are denoted by x i. At steady state and assuming neutrality, we have the following frequencies: x 0 = v v + w and x i = ( w u ) ( v v + w ) ( u v + u ) i. Let us consider a numerical example: w = 10 −7, u = 10 −9, v = 10 −7 per cell division.
Then, cells that have made as many as 10 steps toward the target have a frequency of about 5 × 10 −19 and are present on a planetary scale with a total cell number of the order on 10 30.Most ensuing searches in the regeneration process will immediately lead away from the target and so fail with high probability. The average time until a single search hits the target would be exponential in L. However, the starting condition is regenerated all the time, with unsuccessful searches being discarded.
The result is that we need only polynomially many searches in L to find the target with high probability. That is, the search time is exponential in k but not in L. Sexual reproduction and exchange of genetic material can further increase the efficiency of the search processes by a linear factor.With the regeneration process, a planet of bacteria seems well equipped to discover new functional tools on a time scale that is geologically rapid. Our modeling exercise suggests that as the environment calls for new functions to be advantageous, the relevant sequences might already preexist in the population, either fully or partially formed. The coming together of such sequences in single cells can then lead to genetic machinery able to exploit a new ecological niche. The GOE and its biological consequencesArmed with the preceding results, we can take a closer look at the timing and dynamics of the GOE.
As introduced earlier, the GOE represents the moment in geologic history when O 2 in the atmosphere permanently rose above a threshold of about 10 −5 of its present-day level. There is reason to believe that oxygenation was protracted, with O 2 increasing to levels perhaps comparable to today’s over some 200 million years, before declining to the relatively low values thought to characterize most of the Proterozoic Eon (, ). A growing number of geochemical analyses also suggest that low levels of oxygen built up at least locally and transiently within the water column or in benthic mat communities as early as 3 billion years ago. Thus, available geochemical data indicate that either oxygenic photosynthesis evolved 2.4 billion years ago and rapidly oxygenated the atmosphere and surface oceans (requiring that all geochemical evidence for earlier local oxygenation be interpreted in terms of later oxic diagenesis) or that oxygen consumption matched production for the first several hundred million years of cyanobacterial history. In the latter case, the threshold represented by the GOE would reflect a decreasing supply of oxidants from volcanic gases, hydrothermal fluids, and basaltic rocks; increased rates of oxygenic photosynthesis; increased hydrogen escape from the top of the atmosphere; or some combination of these (, ). Total rates of primary production would have been set by nutrient availability, especially P. Because of adsorption onto iron hydroxides and removal by incorporation into vivianite in anoxic environments, P availability is thought to have been low in Archean oceans, and although somewhat higher in Proterozoic seas, it was still well below Phanerozoic values (–).
In turn, the proportional importance of oxygenic photosynthesis would have reflected Fe/P, with anoxygenic photosynthesis favored as long as electron donors such as ferrous iron were available.Wherever and whenever O 2 first accumulated, life figures prominently in its causation and consequences. Oxygenic photosynthesis is generally accepted as necessary for terrestrial oxygenation , requiring coupled photosystems I and II in all their molecular complexity. Similarly, early oxygenation made the origin and expansion of aerobic respiration, as well as the diversification of oxygen-requiring biosynthetic pathways, possible.
Cyanobacteria are ecosystem engineers: Once O 2 began to accumulate in the surface ocean, alternative electron donors were eliminated.What, then, governed the timetable of the GOE and its biological consequences? Was it influenced most strongly by the time scale on which mutations could result in adaptations for the production and utilization of oxygen? Or does natural selection discover physiological adaptations quickly, shifting focus onto environmental history?
Given the geochemical evidence that cyanobacteria, and thus oxygenic photosynthesis, preceded the GOE by as much as several hundred million years, it is unlikely that the exact timing and dynamics of the GOE are explained by the evolution of oxygenic photosynthesis per se. Instead, we can imagine that there was a particular steady state between the concentration of oxygen and the biomass of cyanobacteria before GOE. The steady-state level of oxygen is given by the rate of production from cyanobacteria divided by the rate of oxygen removal.Let x denote the biomass of cyanobacteria and z the concentration of oxygen in the atmosphere. The time derivative of oxygen concentration z.
Can then be stated in terms of a simple equation, z. = a x − b z, where the rate of O 2 production is proportional to the abundance of cyanobacteria, ax, and the O 2 removal rate is proportional to the abundance of oxygen, bz. The parameters a and b are appropriate rate constants. The steady-state level of oxygen, z = ax/ b, is proportional to the abundance of cyanobacteria. Given this, the GOE could reflect an increase in the abundance of cyanobacteria as they evolved to be better competitors in the global ecosystem. In this case, the ratio z/ x would remain constant, and the rate constants a and b would not change. Another possibility, favored by us, is that the physical environment of the planet changed, leading to a decline in the rate constant, b, for oxygen removal.
This could be caused, for example, by the growth of stable continental cratons, a decline in volcanic emissions of reduced gases and ions, a change in the flux of hydrogen out of the top of the atmosphere, or some combination of these processes. If b declines, then the steady-state level of oxygen increases, but the ratio z/ x also increases. The accumulation of O 2 in the atmosphere and surface ocean would sweep alternative electron donors from most parts of the photic zone. Thus, anoxygenic photosynthetic bacteria dependent on Fe 2+ or H 2S for photosynthesis would become strictly limited—a decline in Fe/P, in the formulation of Jones et al. —permanently favoring primary production by cyanobacteria.The accompanying box and figure present a deliberately oversimplified model for ecosystem change at the GOE. The main inference we draw from this is that whereas rates of oxygen removal might have declined gradually through time, the transition to an oxic atmosphere and surface ocean, with cyanobacteria dominating photosynthesis, would have occurred rapidly once a critical threshold was reached. modeled the GOE as the initial appearance of oxygenic photosynthesis but similarly concluded that the oxic transition was rapid.
Our simple model of bacterial sequence evolution, introduced above, would suggest that shifting redox conditions would quickly be exploited by bacteria with novel physiologies that make use of oxygen in energy metabolism or biosynthesis. Support for this comes from molecular clock estimates of ca. 2310 Ma for the origin of sterol biosynthesis, an oxygen-requiring pathway. A simple model for the GOE.In our deliberately simplified model for the GOE, we consider an ecosystem with two major primary producers: anoxygenic photosynthetic bacteria and cyanobacteria. Anoxygenic photosynthetic bacteria can use a variety of electron donors, but in early oceans, ferrous iron (Fe 2+) would have been most important. Cyanobacteria obtain the electrons needed for photosynthesis by splitting water. Anoxygenic photosynthetic bacteria and cyanobacteria compete over the limiting resource, phosphate.The density of anoxygenic photosynthetic bacteria and cyanobacteria is denoted by x 1 and x 2.
Their time derivates are x. Their reproductive rates are r 1 and r 2. Considerthe following system of equations. 2 = x 2 ( r 2 − c x ) + u 2The density-dependent death rate, − cx, arises from competition over limiting resources and regulates the total abundance, x = x 1 + x 2.
We include small migration rates, u 1 and u 2, from ecological niches, where cyanobacteria and anoxygenic photosynthetic bacteria could exist independently of each other.The reproductive rate of anoxygenic photosynthetic bacteria is multiplied by the concentration of ferrous iron, z 1. As discussed in the main text, oxygen is produced by cyanobacteria and removed by both respiration and geophysical events. For the concentration of oxygen, z 2, we have the equation z. 2 = ax 2 − bz 2, which leads to the steady state z 2 = ax 2/ b. Thus, the steady-state concentration of oxygen is proportional to the abundance of cyanobacteria.
The availability of ferrous iron is reduced by the accumulation of oxygen: For the steady state of ferrous iron, we assume z 1 = 1/(1 + α z 2).Before the GOE, there is negligible oxygen, and thus, in our model, we have z 1 ≈ 1. In this case, anoxygenic photosynthetic bacteria dominate the ecosystem provided r 1 r 2. We model the dynamics of the GOE by assuming that the removal rate of oxygen, b, declines over millions of years. Faily tumbler.
The process leads to a slow but continuous increase in the oxygen concentration, slowly reducing the abundance of ferrous iron. There is a sharp transition when the effective growth rate of anoxygenic photosynthetic bacteria becomes less than that of cyanobacteria, r 1 z 1.
The broader theoretical challenge of Earth’s timetable of evolutionThe GOE played out in a world populated by Bacteria and Archaea, but what about the NOE and beyond? Renewed oxygenation in the Neoproterozoic Era and the temporally associated rise of eukaryotic phytoplankton to ecological prominence could be amenable to modeling broadly similar to that outlined for the GOE. As noted earlier, the pattern of redox change in Neoproterozoic to Early Paleozoic oceans is debated, with estimates for when PO 2 reached 50% PAL ranging from 800 Ma to the Cambrian Period or later. However, in general, full oxygenation of ocean basins appears to have been achieved more than transiently only in the Paleozoic Era (, ). Renewed oxygenation could have been driven by supercontinental breakup, increasing organic carbon sequestration in newly formed and rapidly subsiding basins.
Increased P supply from the weathering of Neoproterozoic large igneous provinces (, ) could also have pushed Earth system to a new redox state, which, in turn, would have reduced iron-based sinks for P. Eukaryotic phytoplankton and macroscopic animals with high demand for oxygen diversified in the context of this change. Phytoplankton radiations may reflect increased nutrient supply , whereas animal radiation likely reflects the consequent increase in food supply and PO 2 increase above a critical metabolic threshold, as well as a decrease in the incursion of anoxic water masses into the surface ocean and possibly feedbacks of animal evolution onto the environment (, ). That is, on the broadest planetary time scale, the physically driven GOE and NOE together appear to have set the timetable of evolution.Phanerozoic diversification of animals, plants, and protists documents continuing evolutionary innovation and biological interactions, and these, in turn, reflect, at least in part, the continuing influence of planetary change for example, see the study of Vrba , including shifting continents, dynamic climates, and occasional transient geophysical or astrophysical perturbations that drive mass extinctions. Rates of genetic adaptation may be most limiting at the times of rapid and pronounced environmental perturbation that mark mass extinction. As is true for Bacteria and Archaea, a theory explaining the fundamental time scale of evolutionary innovation of eukaryotic organisms remains to be developed. Eukaryotic species have smaller population sizes, longer generation times, and a much diminished capacity for horizontal gene transfer.
At the same time, they have distinct mechanisms of genetic regulation, which might both facilitate and constrain the evolution of novel morphologies (, ). Evolutionary innovations in plants and animals reflect the accumulation of characters through time and the ecological circumstances under which character change took place. Angiosperms, for example, postdate the evolution of seed plants by more than 200 million years, their diversification and remarkable ecological success reflecting the developmentally controlled evolution of fruits and accelerated life cycles, as well as functional innovations in leaves and water transport, bolstered by coevolution with animal pollinators and grazers. We suspect that, relative to bacteria, evolutionary timing for plants and animals, fungi, and protists will be governed to a greater extent by the accumulation of complex character combinations and other biological factors, including those that help to define the effective environment of populations (this would also be true for younger bacteria, as eukaryotes became important components of their environments). Nonetheless, the fossil record suggests that eukaryotic evolution has continually been influenced by changes in the physical environment, with rates of genetic discovery sufficiently fast to track most geologically resolvable rates of environmental change.
CONCLUSIONSOn billion-year time scales, the evolutionary dynamics of a planet is fast and its evolutionary potential is vast; the pace of evolution is primarily determined by the physical history of the planet. The timetable of evolution, then, is in no small part determined by geophysical events. In the radical formulation of this view, eukaryotes and macroscopic life emerged when the planet was ready for them. Because stars produce specific chemical elements at distinct phases in their life cycles, it could turn out that (a small fraction of) planets produce specific life forms at distinct phases in their evolution. In contrast, on the time scale of transient environmental perturbations such as those associated with the mass extinctions of the last 500 million years, rates of genetic accommodation may be limiting.In formulating his theory of evolution by natural selection, Charles Darwin was inspired by Charles Lyell’s Principles of Geology , recognizing in Lyell’s view of Earth history the almost limitless expanse of time he thought necessary to understand evolution. In the 21st century, an increasingly well-resolved timetable of evolution presents opportunities for renewed collaboration between geochronology and evolutionary theory. Resolving the theoretical challenges of evolution’s timetable will sharpen our understanding of life’s long evolutionary history while informing continuing astrobiological exploration of our own solar system and beyond.
We are grateful for the path-breaking research of S. We thank two anonymous reviewers for critical comments that improved our paper. Funding: A.H.K.
Acknowledges support from NASA Astrobiology Institute (contract NNA13AA90A). Author contributions: A.H.K. Is primarily responsible for researching the section on calibrating the fossil record, whereas M.A.N. Is primarily responsible for researching the section on evolutionary theory; both authors contributed equally to writing the paper.
Competing interests: The authors declare that they have no competing interests. Data and materials availability: All data needed to evaluate the conclusions in the paper are present in the paper. Additional data related to this paper may be requested from the authors. Schulte P., Alegret L., Arenillas I., Arz J. A., Barton P.
R., Bralower T. J., Christeson G. L., Claeys P., Cockell C. S., Collins G.
S., Deutsch A., Goldin T. J., Goto K., Grajales-Nishimura J. M., Grieve R. F., Gulick S. S., Johnson K. R., Kiessling W., Koeberl C., Kring D.
A., MacLeod K. G., Matsui T., Melosh J., Montanari A., Morgan J. R., Nichols D.
J., Norris R. D., Pierazzo E., Ravizza G., Rebolledo-Vieyra M., Uwe Reimold W., Robin E., Salge T., Speijer R.
R., Urrutia-Fucugauchi J., Vajda V., Whalen M. T., Willumsen P. S.,The Chicxulub asteroid impact and mass extinction at the Cretaceous-Paleogene boundary. Science 327,1214–1218 (2010).
Why Early Access?“The game is still currently in development and with a game based around the community creating and sharing of these creations, early access will allow us to push updates and new features and see how well they are received as well as just further optimizing and finalizing the core game features.”Approximately how long will this game be in Early Access?“The plan is to take hopefully 4 months to find finish up the game’s level editor’s main features, campaign levels, and multiplayer features. After this, future updates will continue to be published adding new features/bug fixes post “release.””How is the full version planned to differ from the Early Access version?“The early access version will slowly introduce new features that aren’t 100% stable, and will slowly come closer to a much more optimized and functional product. As where the full version will have all the features planned finished, optimized, and stable.
Another main difference is the levels created in early access versions might not be compatible with future/different versions while all versions of the released Cranium Conundrum will be compatible with each other and able to play on (as long as the level was made in a released version of the game).”What is the current state of the Early Access version?“The current state of the Early Access version is in early alpha. The game is still going through bug fixes and engine optimizations and is still unstable and prone to crashes and bugs.”Will the game be priced differently during and after Early Access?“We plan to gradually raise the price once the game reaches near it’s finalized state and all planned content and features have been added and finished.”How are you planning on involving the Community in your development process?“The community feedback and involvement will be crucial to the development of this game in its early access state. Bugs and Crashes will be able to reported to help us fix any issues users come across in the alphas. In addition to bug reporting, the community will also be able to submit ideas for features for the level editor mode (e.g. Gimmick ideas, materials, stickers, or even costume ideas) and most suggestions will be taken into consideration during the development of this game.”. About This GameCranium Conundrum is a physics-based puzzle platformer taking place in the dream worlds that you create!
- суббота 11 апреля
- 26